Chapter 1 Introduction
1.1 Cognitive enhancement
As long as they have been in existence, humans have strived to better themselves. This “will to improve” is a fundamental part of human nature (Sloterdijk, 2009). Even more so than other animals, humans are born into this world as “unfinished” animals: we rely on tools and materials to keep ourselves warm, sheltered and fed. But humans also aspire to transcend themselves in a different sense, beyond what is purely necessary for survival. Peter Sloterdijk coined the term anthropotechnics for the collection of tools at our disposal to enhance ourselves, and thereby fulfill our potential (2001). At first, our primary anthropotechnics aimed at cognitive enhancement were psychosocial in nature, consisting mostly of cultural practices, training, and other forms of self-discipline.
In more recent history, technology has started to play an ever-increasing role, and has offered more direct means for cognitive enhancement. Our first penchant was to enhance our cognitive capacities by externalizing them: we expanded our memory through writing systems, improved our numerical cognition through calculators, and used maps to augment our navigational skills. These and other technologies are now so commonplace that some consider them to have become part of our extended mind (Clark & Chalmers, 1998).
Now, we are entering a new age of neuroenhancement (Clark & Parasuraman, 2014), which is characterized by the internalization of technology. With the advent of genetics, pharmacology, and other biological disciplines, humans have become both the subject and the substrate of technology (Stiegler, 1998). Specifically, this family of techniques could allow for cognitive enhancement by directly acting on the organ that gives rise to our cognitive abilities—the brain. Part II of this thesis explores to what extent non-invasive electrical stimulation of the brain (Cohen Kadosh, 2014; Dayan, Censor, Buch, Sandrini, & Cohen, 2013) can be used to enhance cognitive functioning, specifically attentional performance.
1.2 Attention
Out of the myriad of domains that together make up human cognition, attention is a prime target for enhancement (Reteig, Talsma, Schouwenburg, & Slagter, 2017). Attention is itself comprised of many sub-domains (Chun, Golomb, & Turk-Browne, 2011), multiple of which are investigated in this thesis. But attention can be broadly characterized as the ability to focus on what is relevant, and to ignore what is not. Attention is so crucial that we call upon it in every waking moment, as the sheer amount of information available to us far exceeds our capacity to process it. We could not possibly attend to all the smells, sights and sounds that impinge on our senses. Let alone all the thoughts that arise in our mind, about that holiday we enjoyed last week, our plans for the evening, or that we are a bit stressed at the moment. Attention allows us to focus on just a few things at a time, keeping us from being overwhelmed by the others.
Most of the time, attention successfully keeps us on track towards our behavioral goals, by selecting information in accord with those goals, in the face of distraction. But this is no small feat, and attentional processes can go awry in many different ways. For example, inattention is a core component of ADHD (American Psychiatric Association, 2013), and schizophrenia is also characterized by impairments in the control of attention (Luck & Gold, 2008). Likewise, some aspects of attentional function decline with age, such as the prevention of interference by distracting information (McNab et al., 2015).
Even in otherwise healthy individuals, attention does not always work seamlessly. Sometimes we can be so focused on something that we neglect to shift our attention to more important matters, for example when immersed in a phone conversation while driving. Attention can also be so effortful to maintain that it starts to slip away, such as when listening to a boring presentation. In Part III of this thesis, I attempted to investigated why it is so difficult to sustain attention for prolonged periods of time.
1.3 Electrical brain stimulation
The first aim of this thesis (Part II) is to investigate whether such limitations of attention can be overcome. I studied whether non-invasive brain stimulation can be used for this purpose, in the form of transcranial Direct Current Stimulation (tDCS).
In its modern form, tDCS has been in use since around the turn of the century1 (Nitsche & Paulus, 2000; Priori, Berardelli, Rona, Accornero, & Manfredi, 1998). tDCS is applied by placing two electrodes (Figure 1.1A) on the body (at least one of which on the scalp), and passing a small current in between, which flows from the anode (the electrode where current enters the body) to the cathode (the electrode where current exits the body) (Gebodh et al., 2019) (Figure 1.1B). Some of this current will flow across the brain, creating a small electric field which can ultimately increase or decrease neuronal excitability and plasticity (Reato et al., 2019).
Direct current is applied with tDCS, but the stimulation waveform can also consist of alternating current at a single frequency (transcranial Alternating Current Stimulation; tACS) or multiple frequencies (transcranial Random Noise Stimulation; tRNS) (Fertonani & Miniussi, 2017). This family of techniques is sometimes collectively referred to as transcranial Electrical Stimulation (tES) (Gebodh et al., 2019). The current in tES is typically at an intensity of 1–2 milliampere (mA), for 10–30 minutes (Bikson et al., 2016), flanked by two short (< 1 minute) periods where the current is gradually ramped up from or down to zero mA (Figure 1.1C). As a placebo control condition or sham stimulation, the current is often ramped down again soon after the ramp-up phase. This is presumed to produce similar sensations (e.g., itching, tingling), without delivering enough current to affect brain activity (Fonteneau et al., 2019).
The physiological effects of tDCS are complex and not yet fully understood (Jackson et al., 2016; Liu et al., 2018; Pelletier & Cicchetti, 2015; Stagg, Antal, & Nitsche, 2018). tDCS can have both online effects (during the stimulation) as well as offline effects (that outlast the duration of the stimulation). It is clear that the electric field that is produced with tDCS (Figure 1.1D) is not large enough to directly induce action potentials, in contrast to other brain stimulation techniques such as transcranial magnetic stimulation (TMS) (Parkin, Ekhtiari, & Walsh, 2015). But early in vitro (Terzuolo & Bullock, 1956) and in vivo (Bindman, Lippold, & Redfearn, 1964; Creutzfeldt, Fromm, & Kapp, 1962; Landau, Bishop, & Clare, 1964; Purpura & McMurtry, 1965) animal studies observed that tDCS can nonetheless either increase or decrease spontaneous firing rates and evoked potentials. The direction of these online effects strongly depends on the orientation of the electric field (among many other factors) (Bikson, Paulus, Esmaeilpour, Kronberg, & Nitsche, 2019). An anode over the cortical surface (producing an inward current flow) will depolarize the soma of neurons, bringing their membrane potentials closer to the firing threshold. Reversely, a cortical cathode (producing an outward current flow) will hyperpolarize the soma, bringing the membrane potential further from the firing threshold.
These findings were reproduced in the first human studies, which applied anodal and cathodal tDCS to the motor cortex2 (Nitsche & Paulus, 2000, 2001). The motor cortex allows for a direct read-out of the physiological effects of tDCS, by applying a TMS pulse during or after tDCS. TMS can induce motor evoked potentials: slight muscle twitches that can be measured with an electrode on the skin (usually on the arm or hand, depending on the exact region of the motor cortex that was stimulated). When TMS was applied right after anodal tDCS, the motor evoked potentials were larger, meaning tDCS increased cortical excitability in the motor cortex; when TMS was applied right after cathodal tDCS, the motor-evoked potentials were smaller, suggesting that tDCS decreased cortical excitability.
Ever since, anodal tDCS is generally regarded as excitatory, while cathodal tDCS is inhibitory. However, this distinction need not necessarily hold for other brain areas than the motor cortex (Bestmann & Walsh, 2017; Parkin et al., 2015). Nevertheless, many studies have since applied anodal tDCS to other brain areas with the aim to improve brain function (Grossman et al., 2018). Generally, anodal tDCS has indeed been reported to improve behavioral performance on a range of cognitive tasks (Coffman, Clark, & Parasuraman, 2014; Santarnecchi et al., 2015): from learning and memory, to language, and visual perception. The cognitive effects of cathodal tDCS appear to more diffuse (Jacobson, Koslowsky, & Lavidor, 2012).
When the stimulation lasts for more than a few minutes, tDCS may also have offline effects (Bindman et al., 1964; Nitsche & Paulus, 2001), up to at least a few hours. There are multiple candidate mechanisms that could underpin these offline effects, but they in part resemble long-term potentiation/depression-like synaptic plasticity (Bikson et al., 2019). For instance, tDCS after-effects depend on NMDA receptor signaling: changes in excitability are blocked when administering NMDA antagonists (Liebetanz, 2002; Nitsche et al., 2003). Also, both anodal and cathodal tDCS can affect glutamate levels (Clark, Coffman, Trumbo, & Gasparovic, 2011; Stagg et al., 2009).
When stimulation sessions are repeated, the offline effects can last much longer than after a single session of tDCS (Monte-Silva et al., 2013). Several studies that repeatedly paired tDCS with cognitive tasks have reported behavioral effects several weeks or even months after the last stimulation session (e.g., Filmer, Varghese, Hawkins, Mattingley, & Dux, 2016; Looi, Duta, Brem, Brem, & Huber, 2016; Snowball et al., 2013; but see Nilsson, Lebedev, Rydström, & Lövdén, 2017). The prospect of long-term effects is what makes tDCS such an attractive technique for enhancement—and for therapeutic purposes. Indeed, the efficacy of (mostly anodal) tDCS has been investigated for a wide range of neurological and psychiatric disorders (Lefaucheur et al., 2016): from Parkinson’s disease, to depression, and autism (Lefaucheur, 2016).
However, while many of these initial results are exciting, there is also a growing consensus that—in spite of what the literature currently seems to suggest—tDCS cannot be a panacea to enhance every imaginable cognitive skill or treat any mental disorder (Bestmann & Walsh, 2017; Parkin et al., 2015). After two decades of contemporary tES research, our understanding of its neurophysiological mechanisms is still rudimentary (Fertonani & Miniussi, 2017; Liu et al., 2018), which makes it difficult to predict what the behavioral outcome of tES will be (Berker, Bikson, & Bestmann, 2013; Bestmann, Berker, & Bonaiuto, 2015). Recent meta-analyses show that the efficacy of tDCS to enhance cognitive function might not be as large as initially thought (Horvath et al., 2015a; Medina & Cason, 2017). In addition, more failures to replicate tES findings have emerged (e.g., Boayue et al., 2019; Horvath et al., 2015b; Learmonth et al., 2017; Veniero, Benwell, Ahrens, & Thut, 2017), which might partly stem from doubts about the methodological rigor in previous tES studies (Héroux, Loo, Taylor, & Gandevia, 2017; Horvath, 2015; Minarik et al., 2016). This emphasizes the need for work that synthesizes findings across studies (Chapter 2), and further determines the effects of tDCS on cognitive functions, such as attention (Part II).
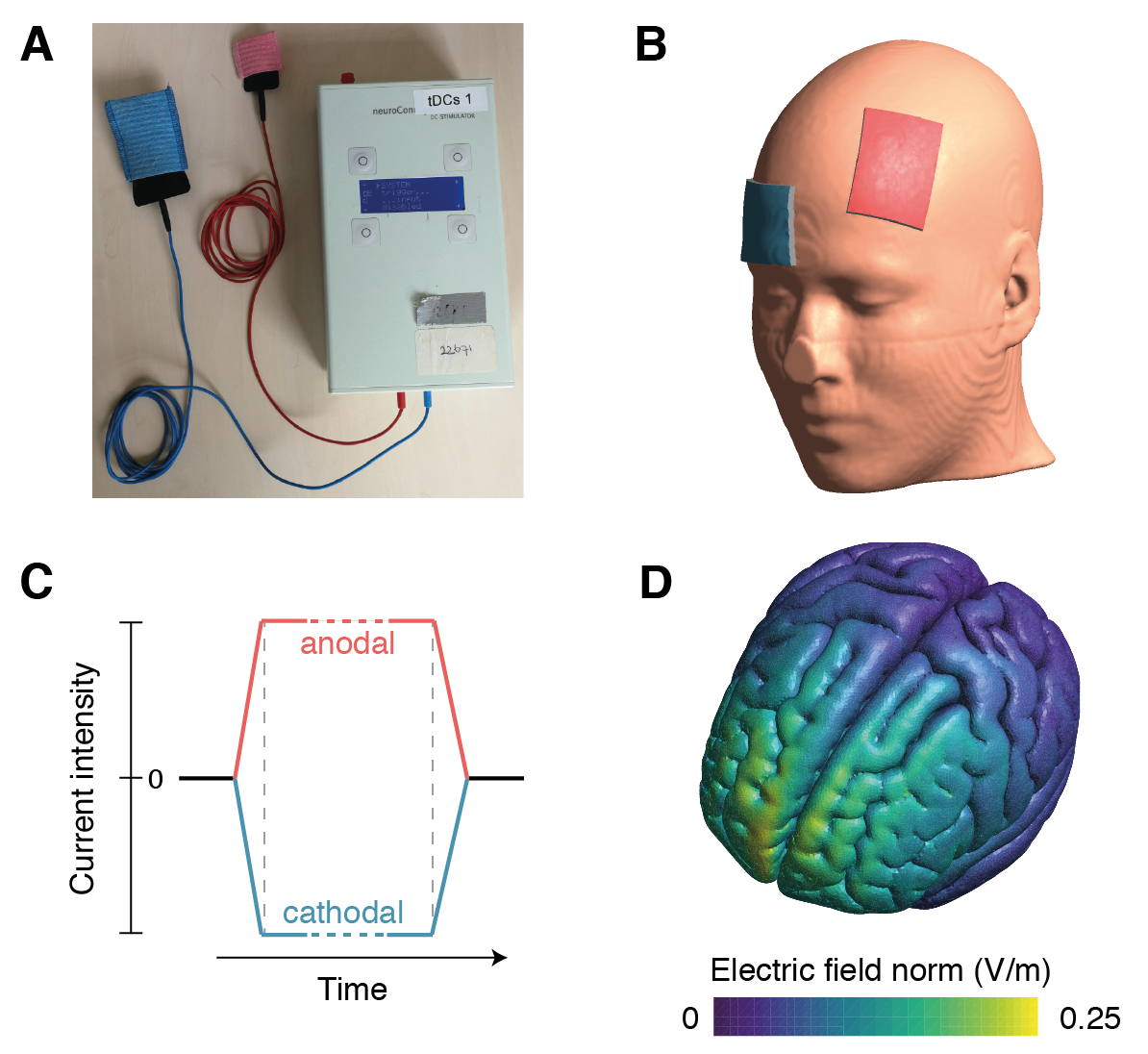
Figure 1.1: transcranial Direct Current Stimulation. (A) The tDCS device (neuroConn DC-STIMULATOR-PLUS) used for the studies in this thesis, connected to a pair of rubber electrodes (in black). Shown are two electrode sizes: 3x3 cm and 7x5 cm, as was used in Chapter 3. To make contact with the skin, the electrodes can either be inserted in sponges with saline solution (as shown in the image), or can be covered in conductive paste (as in the studies in this thesis). (B) tDCS montage that was used in Chapter 4 and Chapter 5. One electrode was placed over the left dorsolateral prefrontal cortex (F3 in the international 10-20 system); the other was placed on the right forehead (approximately corresponding to 10-20 position Fp2). Shown here is the “anodal tDCS” montage with the anode (in red) over F3 and the cathode (in blue) on the forehead. For “cathodal tDCS”, the electrodes were swapped. (C) The tDCS waveform for the anodal (red) and cathodal (blue) electrodes. The dotted lines mark the end of the ramp-up and start of the ramp-down periods (typically < 1 minute), in which the current intensity is gradually increased and decreased, respectively. In between, the current is held at a constant intensity, typically at 1–2 mA for 10–30 minutes. (D) Simulated electric field for the montage in (B), for the gray matter in the MNI152 template, calculated using SimNIBS software (Version 2.2.1; Saturnino et al., 2018; Thielscher, Antunes, & Saturnino, 2015). As is typical with tDCS, peaks in the electric field are localized near the electrodes, but they do not necessarily occur exactly underneath, and the distribution of the field is diffuse.
1.4 Changing attentional performance
Broadly, this thesis is a study of the short-term plasticity of attentional processes: how susceptible is attention to change, and what are the neural processes that underlie such changes? In Part II, I examined whether attention can be improved with tDCS. In Part III, I investigated the “other side of the coin”, i.e. decreases in attention, prompted by prolonged periods of sustained attention.
First, in Chapter 2, I provide an overview of previous studies that have used tES to attempt to enhance attention (until mid-2016), clustered into different sub-domains of attention (visual search, spatial attention, sustained attention, and other studies). This review revealed that the literature until that point was mired in inconsistencies, characterized by a large variability in both study design and outcome. This did not provide a clear foundation for the type of large-scale studies I originally had in mind: to apply tDCS over the course of several sessions, to allow for more long-term effects on attention (c.f. Talsma, Kroese, & Slagter, 2017). Instead, I first decided to try and consolidate previous single-session tDCS results, in two domains of attention: spatial and temporal attention.
1.4.1 Spatial attention
Spatial attention allows us to orient to a certain location in our environment, such as a part of the visual field (Wright & Ward, 2008). Because visual acuity is best at the fovea, shifting visuospatial attention usually involves eye movements (overt orienting) as well. But attention can also be shifted in the absence of eye movements (covert orienting), which still leads to enhanced sensitivity to the part of the visual field that is now in the focus of attention (Carrasco, 2011).
Shifts in spatial attention to a certain location heighten neural activity in corresponding retinotopic regions (Desimone & Duncan, 1995; Kastner, Pinsk, Weerd, Desimone, & Ungerleider, 1999; Tootell et al., 1998; Worden, Foxe, Wang, & Simpson, 2000), such that stimuli in this location can be more easily detected or discriminated. This process is coordinated by a network of frontoparietal brain regions, including the frontal eye fields (Corbetta & Shulman, 2002), which are activated during both covert and overt attentional orienting (Grosbras, Laird, & Paus, 2005; Nobre, Gitelman, Dias, & Mesulam, 2000). Non-human primate studies have shown that sub-threshold microstimulation of the frontal eye fields causes a shift in visuospatial attention; supra-threshold microstimulation also causes the monkey to make an eye movement (Moore & Fallah, 2001).
Several studies have attempted to affect spatial orienting with tDCS, mostly over the parietal cortex (see Chapter 2). But the results are mixed: while some found they could enhance spatial attention for the visual hemifield contralateral to the stimulated hemisphere, others reported no effects or even decreases in attention. In Chapter 3, given the key role of the frontal eye fields in spatial orienting, I examined whether tDCS over the frontal eye fields could improve performance on a prosaccade task, where participants had to make eye movements to a target as fast as possible. I reasoned this would constitute a fairly direct test of whether spatial attention can be readily affected with tDCS, because there is a clear physiological mechanism that tDCS can act on. Activity in the frontal eye fields ramps up to a threshold, after which a saccadic eye movement is initiated (Hanes & Schall, 1996). If anodal tDCS can increase excitability of the frontal eye fields, this threshold should be reached sooner, thereby decreasing saccadic latency. Such changes can be directly read-out by measuring eye movements with an eye tracker. One earlier study showed that anodal tDCS over the frontal eye fields might indeed speed saccade latency (Kanai, Muggleton, & Walsh, 2012; but see Chen & Machado, 2017).
1.4.2 Temporal attention
Aside from the spatial domain, attention can also be deployed across time. Temporal attention can be isolated using rapid serial visual presentation (RSVP) tasks—where multiple stimuli are presented quickly after each other at the same location. When tasked to search for two or more targets in such an RSVP stream, the first target is usually seen, but the second target is often missed (when it follows the first within about 500 ms). This phenomenon is known as the attentional blink (Raymond, Shapiro, & Arnell, 1992).
In Chapter 4 and Chapter 5, I examined whether tDCS over the left dorsolateral prefrontal cortex (lDLPFC) can decrease the size of the attentional blink. The lDLPFC is one of the brain areas that has been most frequently targeted with tDCS (Santarnecchi et al., 2015), and is also strongly implicated in the attentional blink (Hommel et al., 2006; Slagter, Johnstone, Beets, & Davidson, 2010).
London & Slagter (2015) were the first to study the effects of anodal or cathodal lDLPFC-tDCS on the attentional blink. They found no group effect, but an interesting pattern of individual differences in the response to anodal and cathodal tDCS. Specifically, those that tended to improve their performance following anodal tDCS (i.e., their attentional blink size decreased), tended to worsen during cathodal tDCS (and vice versa). This finding is consistent with earlier studies that find large individual differences in both the AB (Willems & Martens, 2016) and tES effects (Krause & Cohen Kadosh, 2014; L. M. Li et al., 2015). Individual differences in tES effects could stem from a range of sources, including differences in neuroanatomy, baseline brain state, or demographic and other factors.
Since London and Slagter’s (2015) finding was both unprecedented and based on an exploratory analysis, in Chapter 4 I determined to what extent I was able to replicate this result. In Chapter 5, I aimed to identify potential drivers of these individual differences. Specifically, I examined spontaneous eye blink rate (sEBR) as a proxy for striatal dopamine levels, as dopamine may mediate both the attentional blink (Jongkees & Colzato, 2016) as well as the physiological effects of tDCS (Stagg et al., 2018).
1.4.3 Sustained attention
The attentional blink clearly demonstrates that attention is fallible, but this deficit only shows under very specific circumstances. Instead, the limits to attention are more commonly experienced when it has to be sustained for a prolonged period of time. As anyone will attest after a long day of work, sustaining attention is mentally fatiguing (Ackerman, 2011; Hockey, 2013) and cannot be done indefinitely.
Sustained attention could thus be an interesting target for tES: perhaps its time span can be lengthened by enhancing the attentional processes that are called upon. This presupposes that we know which aspects of attention change over time, along with their neural correlates. In Chapter 6, I attempted to shed more light on fluctuations in sustained attention by conducting an experiment on the vigilance decrement.
The vigilance decrement (also known as the time-on-task effect) is observed when people are tasked to monitor incoming information and detect rare, but critical signals. This is surprisingly effortful, and performance on such tasks already starts to decrease after only a few minutes (Warm, Parasuraman, & Matthews, 2008). The vigilance decrement is robust and has been studied for decades, starting from interests in the performance of military radar operators (Mackworth, 1948). Decrements in sustained attention also loom in many other situations, including long stretches of driving, traffic control, or quality inspection. Nonetheless, still little is known about the underlying neural mechanisms. Also, there remains considerable debate on the ultimate cause of the vigilance decrement: does performance decrease due to true exhaustion, or do people instead succumb to the likes of boredom, mind wandering, or decreasing motivation towards the task? I attempted to address these issues by tracking changes in attention-related brain activity during performance of a vigilance task, using electro-encephalography (EEG).
1.5 Measuring attention with EEG
EEG is a direct measure of the post-synaptic activity of large groups of neurons (thousands to millions) (Cohen, 2017). In human research, EEG is one of the most frequently used techniques to relate brain activity to cognitive functions. One way is to compute event-related potentials (ERPs) (Luck, 2005): the averaged EEG response time-locked to a certain event (e.g., a visual stimulus). In addition, the EEG consists of neural oscillations: rhythmic fluctuations in a certain frequency band, reflecting changes in neuronal excitability (Buzsáki & Draguhn, 2004; Cohen, 2014). Both ERPs and oscillations can be used to measure attention. The amplitude of early visual ERPs can change depending on whether the stimulus that elicits them is attended or not (Luck et al., 1994; Mangun & Hillyard, 1991; Slagter et al., 2016). Similarly, the power and phase of neural oscillations also covary with attention, particularly in the alpha (ca. 8–12 Hz) and theta (ca. 4–7 Hz) bands (Clayton, Yeung, & Cohen Kadosh, 2015; Diepen, Foxe, & Mazaheri, 2019; Klimesch, 2012).
In Chapter 6, I used these different EEG-based measures of attention to investigate which stage of attentional processing is most affected during the vigilance decrement. In Chapter 5, I also used EEG to record sEBR, as movements of the eye lids also create electrical signals that can be picked up with EEG electrodes.
1.6 Overview
To recapitulate, this thesis studies the neuroplasticity of attention from two different perspectives. In Part II (Chapters 3, 4 and 5), I used tDCS with the aim to enhance attentional performance. In Part III (Chapter 6), I conducted an EEG study to examine decreases in attentional performance, when attention is sustained for a prolonged period of time. The data, code and materials for all Chapters in this thesis are openly available (see the overview of online resources in Appendix D, or follow the links contained in each respective chapter).
In the next chapter (Chapter 2), I present a literature review of previous work on tES and attention (until mid-2016). I examined studies that paired tES with visual search tasks, spatial attention tasks, sustained attention tasks, and other types of tasks. Overall, the evidence that tES is an effective tool to enhance attention was mixed.
The studies presented in Part II were guided by the insights from the literature review in Chapter 2. First (Chapter 3), I applied tDCS over the frontal eye fields during an eye movement task, to try to more conclusively demonstrate that tDCS can be used to enhance (overt) spatial attention (in line with a previous study by Kanai et al., 2012). In the next two chapters, I applied tDCS to the dorsolateral prefrontal cortex, with the aim to enhance temporal attention. In Chapter 4, I attempted to replicate a previous finding (London & Slagter, 2015) showing that tDCS is able to affect the size of the attentional blink, but that the exact effects of tDCS differ across individuals. In Chapter 5, I examined how dopamine relates to the attentional blink (using sEBR as a proxy for dopamine), and whether dopamine levels can explain the individual differences in the response to tDCS.
To foreshadow my findings, I was unable to replicate the results reported by Kanai et al. (2012) and London & Slagter (2015). In general, I found no compelling evidence for any effects of tDCS in any of the chapters in Part II. These findings add to the current controversy in the field regarding the efficacy of tES (Bestmann & Walsh, 2017; Héroux et al., 2017; Horvath et al., 2015b). Possible explanations for and implications of these null results are discussed in more detail in the discussion section of each chapter, as well as in the general discussion in Part IV of this thesis.
In Chapter 6 in Part III of the thesis, I used EEG to track changes in attention during prolonged performance of a sustained attention task. I show that decreases in behavioral performance of the task are partly—but not fully—explained by motivation. Further, the timing of the EEG response to visual stimuli became more variable as task performance decreased, suggesting that attentional stability is primarily affected in the vigilance decrement. In light of these findings, I also discuss whether tES could potentially be used to counter the vigilance decrement (in Chapter 2, and in the general discussion in Part IV).
However, note that this was preceded by some clinical trials in the mid-20th century (e.g., Redfearn, Lippold, & Costain, 1964). In general, the use of direct current to stimulate the body dates back to the invention of voltaic cells in the 18th century, and even before, when electrogenic species of fish were used for this purpose (Guleyupoglu, Schestatsky, Edwards, Fregni, & Bikson, 2013; Priori, 2003; Sarmiento, San-Juan, & Prasath, 2016).↩
The term “anodal tDCS” is used when the anode is placed over the brain area of interest, and the cathode is placed elsewhere (and vice versa for “cathodal tDCS”). The electrode over the brain area of interest is also sometimes referred to as the “active electrode”; the second electrode is then the “reference electrode”. However, all of these terms can be misleading. The “reference electrode” is not inactive; there are always two “active electrodes”: one anode and one cathode. Also, because a circuit has to be closed for current to flow, current will always enter the brain at one location and exit at another. So “anodal tDCS” or “cathodal tDCS” cannot be applied in isolation—the opposite polarity is always concurrently applied somewhere else.↩